Cells can apply and sense forces to communicate and coordinate with each other. Scientists and engineers have teamed up under the mechanobiology banner to study these cellular forces
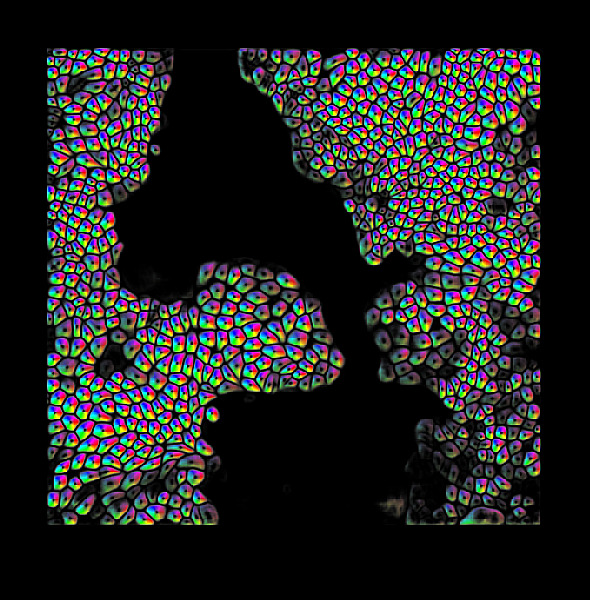
The outermost and the innermost surfaces of any organ are lined by the ‘epithelium’, a tissue composed of cells arranged in a sheet. For instance, the part of the skin that is visible to us, and the inner surface of the lung exposed to airflow, are both lined by epithelia. Their main function is to protect organs from external particles and germs. Therefore, cells of the epithelia are closely packed and are connected with their neighbours through proteins. They latch onto a jelly-like surface called the ‘substrate’ that contains an assortment of proteins. In addition, special proteins jutting out of one epithelial cell can bind to similar ones from the neighbouring cells, like holding hands, allowing them to stick to each other. Similar to pulling someone by their hand, cells use these proteins to apply forces on their neighbours, called ‘cell-cell forces’. Cells also crawl over their substrate by applying forces on it, like the traction of the wheels of a moving car on the road. Since cells and tissues are microscopic, measuring forces acting upon or exerted by them can be tricky. Understanding such mechanical forces is important in the study of cellular processes, such as cancer, fertilisation and wound-healing. ‘Mechanobiology’ is an exciting interdisciplinary field that brings biologists, physicists, mathematicians and engineers together to study forces at the cellular level.
When our skin gets a cut, cells at the site of the wound are scraped away, creating a gap in the epithelial sheet. The cells surrounding the wound coordinate and move together to close the gap. Cells also move collectively when organs are being formed, when the baby is still in the womb of the mother. In the 1960s, several studies observed that the leading edge of a group of moving cells had finger-like protrusions in the direction of movement. This led embryologists and cell biologists to hypothesise that the cells at the leading edge were applying force and pulling the cells behind them. But this idea remained unverified for decades due to the lack of experimental techniques to measure cell-cell forces in tissues. In the late 1990s, Micah Dembo, a theoretical biologist turned mathematician, devised a technique called Traction Force Microscopy (TFM) to measure single-cell forces. In TFM, cells are grown on top of gels containing tiny micron-sized fluorescent beads. This gel is elastic, like a spring. When moving cells apply a force on it, the fluorescent beads in the gel move too. The movement of the beads on the gel can be compared to the movement of an object attached to a spring. Using the stiffness of the gel and the displacement of the beads, we can calculate the force applied by the cell on the gel. Dembo’s group first reported the movement of cells from a soft substrate to a stiff substrate. Using TFM, they showed that cells exert higher forces on stiffer substrates. Due to the force imbalance between soft and stiff substrates, cells move toward the latter.
‘Mechanobiology’ is an exciting interdisciplinary field that brings biologists, physicists, mathematicians and engineers together to study forces at the cellular level
Dembo’s method, however, involved extensive calculations which took hours on a supercomputer. James Butler, a particle physicist, simplified the calculations using a specialised mathematical method so that they could be done in seconds on a laptop. And yet, a decade later, biologists were still struggling to measure forces between cells in a tissue, and again, physicists solved their problem.
In 2009, Xavier Trepat and James Butler improved TFM and came up with Monolayer Stress Microscopy (MSM). When they used this technique to look at wound healing by growing cells on top of gels, the results were bizarre. They expected to see either a uniform distribution of forces across the layer of cells, or higher forces at the leading edge near the wound, and little to no forces in the bulk of the cells. But they observed a mosaic distribution of forces in different directions with no apparent pattern in the bulk of cells.
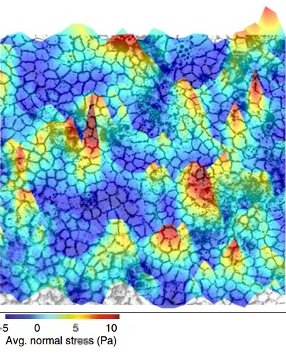
Forces fluctuating with space and time in the epithelial tissue (Image courtesy: Medhavi Vishwakarma)
In 2011, they collaborated with the physicist David Weitz and noted an interesting parallel between epithelia and soft matter. Similarities between soft matter and epithelium were reported for the first time in 2001. Ben Fabry, an electrical engineer, used magnetism to study cell forces. After getting some curious results, he knocked on David Weitz’s doors, who first noticed the similarity. Soft matter refers to materials whose constituents are not ordered. Substances such as coffee beans and foam come under the umbrella of soft matter. Such substances are associated with a peculiar property called jamming/unjamming. A material is said to be ‘jammed’ when the movement of its constituents is constrained by their neighbours, similar to a traffic jam. The epithelium is like soft matter – its constituents, the cells, are deformable. This allows it to protect the body from external agents by forming a continuous barrier. When there is a wound, however, the epithelial tissue ‘unjams’, becomes more fluid, and flows in to fill the gap. As the wound closes, certain cells at the leading edge actively apply force and pull the cells behind them. These specialised cells are called ‘leader’ cells. Medhavi Vishwakarma, Assistant Professor at the Centre for BioSystems Science and Engineering (BSSE), IISc, studies how leader cells are chosen. Cellular forces fluctuate with space and time in the epithelial tissues. These fluctuations dictate leader cell formation. Just like in animal societies, leader cell formation in epithelial cells is decided by democratic, collective decision making.
Epithelia are ‘viscoelastic’ – they are viscous like honey and can flow in the timescale of hours, but are also elastic like spandex, in the timescale of minutes. But why are epithelial tissues viscoelastic? “Epithelial tissues are like a community,” explains Medhavi. “They are connected by cell-cell contacts. When one member (cell) experiences stress, it can be passed on to the other members. Therefore, viscoelasticity at the cellular level can be translated into viscoelasticity at the tissue level.” To understand how solid tissues can flow, we need to zoom in from tissues to micron-sized cells.
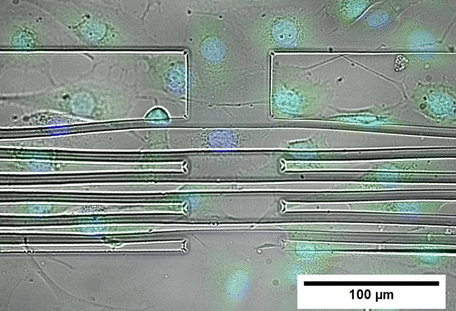
To measure the mechanical properties of adherent cells, Ananthasuresh’s team has devised a specialised substrate with grooves. On stretching the substrate, the cells grown on it are also stretched. Conversely, during cell migration, cells also apply forces on the substrate to sense the stiffness and viscosity of their environment. By measuring the substrate displacement using microscopy, the force applied by the cells can be measured.
GK Ananthasuresh, Professor at the Department of Mechanical Engineering, also studies the mechanics of cells. He studies the mechanics of flexible objects and designs, and makes micromachined devices. “It occurred to me that I am working on things that are small and flexible, and the biological cell is one of those. We were making miniature tools to understand small things, and we wanted to make tools to understand biological cells; like microscopic fingers to hold and handle cells,” says Ananthasuresh. His lab makes ‘mini fingers’ using a polymer called SU-8. Using these, researchers can probe cells and feel how soft or hard they are.
The idea is that biochemical changes inside the cell would reflect in changes in its mechanical response to these ‘fingers’. If these mechanical responses can be measured, then it is possible to predict the biochemical changes that caused them without doing complicated biochemical tests. For instance, a typical eukaryotic cell has a nucleus. The shape and location of the nucleus are perceptibly distinct for different cell types in different conditions. Specific proteins hold the nucleus in place and maintain its shape. In most cells, the nucleus is easy to observe. Hence, by analysing the shape of the nucleus, the researchers can predict the cell type and the protein distribution in that cell, even without biochemical tests. “Biologists are used to measuring parameters directly from experiments. As engineers, we try to deduce parameters that are easy to measure, and from those, calculate the more complex required parameters,” says Ananthasuresh.
By analysing the shape of the nucleus, researchers can predict the cell type and the protein distribution in that cell, even without biochemical tests
At the other end of the campus, Ambarish Ghosh, Professor at the Centre for Nano Science and Engineering (CeNSE), works on heterogeneity inside and outside cells. “Biological materials are so heterogeneous. (Even) at the length of 10 microns, they have several different components and functions,” he says. A physicist by training, his interests include colloidal systems and soft matter physics. In colloidal systems, microscopic particles of one substance are spread throughout another substance, like fat particles suspended in milk. However, these systems are not uniformly dispersed, so if we want to understand their mechanical properties such as density, it is not enough to measure it only at one location. For this, we need tools which can take local readings at different points. Conventional tools such as the ‘rheometer’ can only take an average measurement for the whole sample. “Basically, in analysing the khichdi, you’re not differentiating between the rice and the dal,” explains Ambarish.
To overcome this problem, Ambarish’s group uses tiny positively charged robots which can move around and measure mechanical properties at different locations of the colloidal system. They move like corkscrews when a small magnetic field is applied and so are called ‘helical swimmers’. These swimmers can enter cells and swim inside them.
Using the swimmers, the researchers measured variations in mechanical properties, such as stiffness inside the cells. These swimmers were also used to probe the environment outside the cells, where they were allowed to move randomly in a culture dish containing a mix of normal cells and cancer cells. Surprisingly, the researchers noticed that the swimmers seemed to be trapped near the cancer cells but not near the normal cells. They found that only cancer cells secreted negatively charged acid into their external environment. Therefore, due to electrostatic interactions, the positively charged helical swimmers were stuck near them. “Not only are we taking measurements, but because the electrostatic and mechanical properties change (around a tumour), we are in a way targeting cancer cells. This can have tremendous implications for cancer imaging and drug delivery,” says Ambarish.
“Not only are we taking measurements, but because the electrostatic and mechanical properties change (around a tumour), we are in a way targeting cancer cells”
The reason why the environment inside cells is not homogenous, as Ambarish pointed out earlier, is because the inside of the cell is packed with proteins, carbohydrates and fats of different sizes and stiffness. We need to zoom in further from the micrometre-sized cells to nanometer-sized proteins. Techniques such as Förster Resonance Energy Transfer (FRET) allow us to calculate the tension in a protein molecule. For example, the protein Talin is like a spring. Talin can exist in two forms: extended and compressed. In the compressed form, the two ends of Talin are close to each other, and in the extended state, they are far apart. In FRET, the two ends of a protein are tagged with fluorescent molecules using genetic manipulation. When the protein is compressed, the two fluorophores come close to each other, and interact to give a fluorescent signal. The greater the fluorescent signal, the more the compression in the protein. Talin is normally present in the closed form near the cell membrane. When an external force is applied on the cell, Talin opens up to the extended form, which can be visualised by FRET.
Proteins inside the cell can assemble or break down or change their form dynamically. Therefore, their mechanical properties such as elasticity keep changing with time, making proteins also viscoelastic. Viscoelasticity at the protein level leads to viscoelasticity of cells and subsequently to viscoelasticity of tissues. This explains how tissues can flow like a fluid to heal wounds even though they are solid under normal conditions.
Mechanical changes in cells can trigger biochemical changes and vice versa. “‘Chem’ and ‘Mech’ are in fact anagrams,” chuckles Ananthasuresh. Mechanobiologists are primarily interested in the mechanical properties of cells, while cell biologists are mostly concerned with biochemical pathways in cells. The way ahead in mechanobiology is to understand the crosstalk between mechanical forces and biochemical pathways, according to Medhavi and Ambarish.
It may soon be possible to detect diseases by measuring the mechanical properties of tissues and cells. A familiar example of a change in mechanics due to disease is the stiffening of the breast with breast cancer. Scientists are also trying to detect malaria by estimating the change in the stiffness of infected red blood cells. Mechanical tools and treatments are also finding their way into healthcare settings. An IISc-based startup called SpOvum uses mechanobiology to improve the success rate of in vitro fertilisation (IVF). In IVF, fertilisation of the ovum by the sperm happens outside in a Petri dish. After fertilisation, the embryo grows in the lab until it forms a ball of cells called the ‘blastocyst’. A crucial step in the IVF process is biopsying the blastocyst – a few cells are removed from the ball of cells to analyse the health of the blastocyst. Handling the blastocyst with a micropipette may sometimes damage the blastocyst during biopsy. SpOvum has devised robots to hold blastocysts and handle them during biopsy, reducing damage to the blastocyst. “The field is moving towards ‘mechanodiagnostics’ – diagnosing diseases using mechanical responses,” says Ananthasuresh. We are headed in the direction of Richard Feynman’s vision of ‘swallowing the surgeon’, or nanobots performing surgeries and replacing surgeons.
Sindhu M is a PhD student in the Centre for BioSystems and Engineering (BSSE), IISc, and a science writing intern at the Office of Communications, IISc